Biocatalysts for a more sustainable industry
2018/06/11 Lide Arana Urbieta - Biokimika eta Biologia Molekularra saileko irakasleaEHU Iturria: Elhuyar aldizkaria
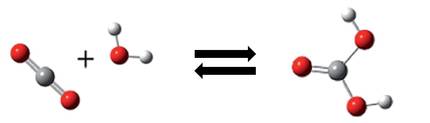
In fact, we would need years to achieve processes that occur every second in our cells. It is wonderful how the speed of any reaction changes when it occurs in our body. For example, a continuous reaction of red blood cells to transport CO2 in our blood, the dehydration of bicarbonate (Figure 1), occurs millions of times more slowly in the middle [1].
What is our secret? How do we get that speed? Living beings form molecules responsible for accelerating or catalyzing reactions, called biocatalysts or enzymes. Although they are not synonymous, most biocatalysts are enzymes, i.e. proteins with catalytic activity.
For each process there is a specific enzyme that catalyzes one and a single reaction (to use a single type of reagent and create a single product), so an enzyme cannot produce two products. They are the most efficient and powerful catalysts known (much more powerful than any inorganic catalyst) and, although we use them since ancient times, the development experienced in recent years has made them today molecules with many applications. We use them to improve the industrial production of different products of daily life.
Around us are many examples of industrial use of enzymes, which have almost unconsciously entered the market. Some are used for industrial food treatment, such as lactose-free milk production. Another known use is the measurements used in quality controls, most of which are based on the activity of some enzyme. In addition, in the pharmaceutical industry, enzymes can be used to obtain a more specific production of drugs. Enzymes use a single substrate to form a single product, so molecular mixtures (stereospecific) that are generated in a chemical synthesis are avoided, avoiding in some cases dangerous secondary products. However, the broader scope of application focuses on improving the sustainability of industrial processes for the greenest generation of chemicals (through non-polluting processes under biological conditions). In addition, numerous enzymes are produced that can help improve washing processes (bleach detergents in cold waters) or mitigate environmental pollution.
However, in nature there are not always enzymes that catalyze processes of interest. As has been said, each enzyme knows a single type of substrate, providing a unique and specific product, and various reactions of industrial interest do not occur in living beings. Therefore, we have started to create new enzymes or improve the ones we had, taking advantage of the knowledge of enzymes and available technologies. So far, the most common route has been to optimize the enzymes we already knew. As is done for the improvement of fruit or plants, mutations in proteins are produced and enzymes of preferred characteristics are selected. This methodology is called corrected evolution. Sometimes mutations are made specifically at certain points, in which case a rational design is said to have been used. However, the design of new enzymes from the vacuum is becoming increasingly a methodology that theoretically would greatly extend the possibilities of obtaining new biocatalysts [2].
As it is essential to understand the design process of a machine, in order to synthesize a suitable biocatalyst it is necessary to fully understand its operation and structure. And what exactly do we know about these molecules?
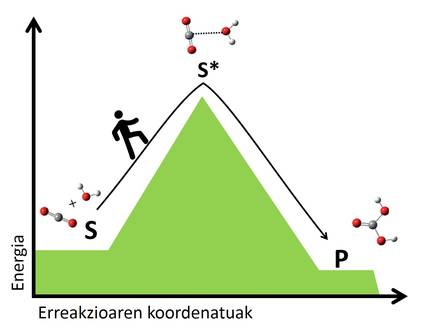
According to the transitional state theory, in all reactions the atoms of the reagents are rearranged, breaking the bonds and forming new molecules. This reorganization creates transition states in which intermediate atomic formations of reagents and products are formed. Molecules in transition are generally unstable and require a lot of energy to reach a transition state from reagents. The greater the energy leap to the transition state, the slower the reaction. It is like passing from one valley to another, the higher the pass, the longer the journey (Figure 2).
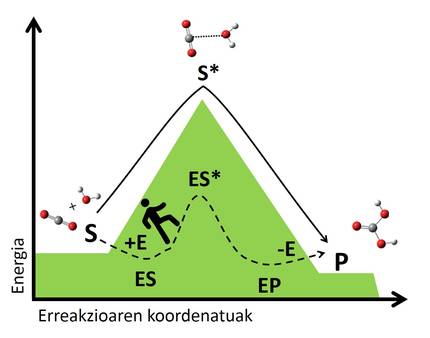
The mechanism of biocatalysts is based on the stabilization of these transition situations through interactions with the substrate. The enzyme binds to the substrate forming a complex that creates a new non-existent pathway of reaction (Figure 3). The complexity is more stable than the system formed by the enzyme and the free substrate, and this stability reduces the energy jump needed to reach the transition state, leading to a faster reaction pathway. Following the example above, we could understand how to find a path that crosses us from one valley to another, since it is a path that requires us less energy than going up and down to the top, so we will arrive faster to the other side.
Thus, if we wanted to design an enzyme to catalyze a reaction, we would need a structure compatible with the transition state of the reaction: something capable of completing molecule stabilizing interactions, which would serve to stabilize the complex and accelerate the reaction. In order for this to occur correctly, in addition to selecting the appropriate atoms to complete interactions with the substrate (with its specific chemical characteristics), atoms must be located in space in a specific position and angle, forming a specific three-dimensional network [3].
In general, the catalysis zone (catalytic zone) is very small compared to the total volume of the molecule. As an example, we can analyze the enzyme that catalyzes the hydration of CO2, carbonic anhydrase (Figure 4). As can be seen, the area of interaction with the substrate is very small if the volume of the entire enzyme is taken into account.
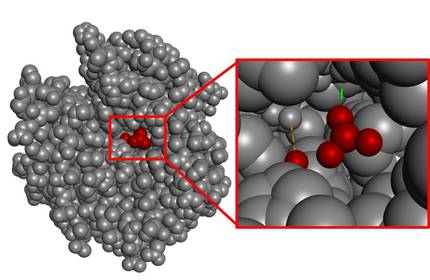
It is known that nature never wastes energy. Consequently, the rest of the structure that is not the catalytic space should not be understood as a surplus, but as an indicator of the complexity involved in achieving the perfect Catalan space. In fact, possible changes in the atom further away from this structure could also destroy the catalytic zone. In other words, the entire structure is essential for the enzyme to be active and it is a complex process to start designing such large molecules from scratch.
In this sense, the development of bioinformatics and structural biology has contributed significantly. Today, quantum mechanics can calculate the transition state of a reaction, simulate the compatible active zone and design the protein structure that would be necessary to complete the structure.
This methodology has allowed catalyzing reactions without known biocatalyst and verifying that it is possible to carry out this process. In addition, these techniques have helped broaden our knowledge about the structure of proteins. However, the catalytic characteristics of these examples obtained do not reach the level of activity of natural enzymes, usually have a reduced catalysis capacity [4].
Consequently, although there is much to improve, the design of new biocatalysts allows us to control the speed of any process of our interest. These techniques allow industrial processes to be carried out at lower temperatures, without the need for organic solvents, with better efficiency, with less water and with less polluting waste. Therefore, we can design the nanombres of life so that industrial processes are compatible with life.
Bibliography
[1] Albert L. Lehninger, David L. Nelson and Michael M. Cox. Fundamentals of Biochemistry (2008), University of the Basque Country, 1st edition.
[2] Po-Ssu Huang, Scott E. Boyken and David Baker. - Nature (2016), 537 (7620):320-7.
[3] Karlheinz Drauz, Harald Gröger and Oliver May. Enzyme catalysis in organic synthesis (2012), Wiley-VCH.
[4] Kiss G, Çelebi-Ölçüm N, Moretti R, Baker D, Houk KN. Computational enzyme design. Angewandte Chemie International Edition in English (2013), 52(22):5700-25.
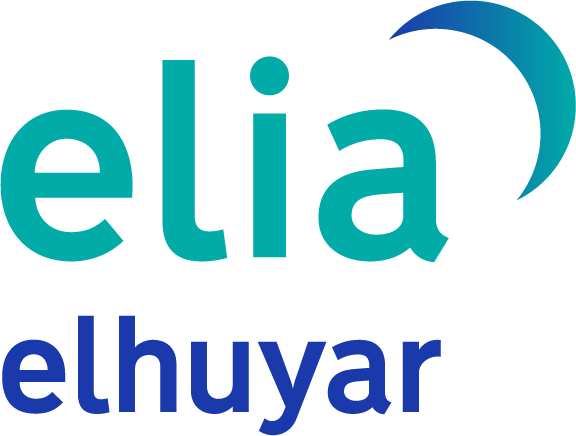
Gai honi buruzko eduki gehiago
Elhuyarrek garatutako teknologia